Silicon-chip-based ultrafast optical oscilloscope: With the realization of faster telecommunication data rates and an expanding interest in ultrafast chemical and physical phenomena, it has become important to develop techniques that enable simple measurements of optical waveforms with subpicosecond resolution.
State-of-the-art oscilloscopes with high-speed photodetectors provide single-shot waveform measurement with 30-ps resolution. Although multiple-shot sampling techniques can achieve few-picosecond resolution, single-shot measurements are necessary to analyse events that are rapidly varying in time, asynchronous, or may occur only once. Further improvements in single-shot resolution are challenging, owing to microelectronic bandwidth limitations.
To overcome these limitations, researchers have looked towards all-optical techniques because of the large processing bandwidths that photonics allow. This has generated an explosion of interest in the integration of photonics on standard electronics platforms, which has spawned the field of silicon photonicsand promises to enable the next generation of computer processing units and advances in high-bandwidth communications. For the success of silicon photonics in these areas, on-chip optical signal-processing for optical performance monitoring will prove critical.
Beyond next-generation communications, silicon-compatible ultrafast metrology would be of great utility to many fundamental research fields, as evident from the scientific impact that ultrafast measurement techniques continue to make. Here, using time-to-frequency conversion via the nonlinear process of four-wave mixing on a silicon chip, we demonstrate a waveform measurement technology within a silicon-photonic platform. We measure optical waveforms with 220-fs resolution over lengths greater than 100 ps, which represent the largest record-length-to-resolution ratio (>450) of any single-shot-capable picosecond waveform measurement technique.
Our implementation allows for single-shot measurements and uses only highly developed electronic and optical materials of complementary metal-oxide-semiconductor (CMOS)-compatible silicon-on-insulator technology and single-mode optical fibre. The mature silicon-on-insulator platform and the ability to integrate electronics with these CMOS-compatible photonics offer great promise to extend this technology into commonplace bench-top and chip-scale instruments.
Several established nonlinear optical techniques exist to measure optical waveforms with few-femtosecond accuracy, but have limited single-shot record lengths of tens of picoseconds and limited update rates. To span the temporal region between electronic measurement and these methods, and to allow for rapidly updateable direct optical detection, techniques have been developed using the space-time duality of electromagnetic waves and related phenomena.
This duality relies on the equivalence between the paraxial wave equation, which governs diffractive propagation of a spatial field, and the scalar wave equation, which governs dispersive propagation of a temporal field. The duality implies that spatial optical components such as a lens or prism have temporal counterparts known as a time-lens or time-prism, which can be implemented by imparting a quadratic or linear temporal phase shift, respectively, to the temporal field. Furthermore, these components allow for temporal processing in a manner analogous to that of the spatial counterparts, such as temporal imaging of the waveform.
Two methods using the space-time duality can be applied to measure ultrafast optical waveforms. Much like a spatial lens can magnify an image, a temporal lens can lengthen an ultrafast waveform in time, allowing for measurement using a photodetector and an oscilloscope that would have insufficient temporal resolution for the unmagnified waveform. This technique is known as temporal magnification.
The second measurement method utilizes the Fourier property of a lens—an object positioned at the front focal plane of a lens will produce a Fourier transform of the object at the back focal plane (Fig. 1a). As the Fourier transform of a temporal waveform is its optical spectrum, extending the spatial Fourier processor to the temporal domain yields a device that converts the temporal (spectral) profile of the input to the spectral (temporal) profile of the output (Fig. 1b). Thus, a measurement of the spectrum at the Fourier plane directly yields the temporal amplitude of the incident waveform, and this process is termed time-to-frequency conversion.
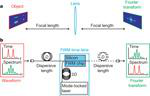
The phase shift for temporal imaging devices is typically applied using an electro-optical phase modulator, but an alternative scheme can be realized by using a parametric nonlinear wave-mixing process such as sum-frequency generation and difference-frequency generation.
This latter technique is called parametric temporal imaging22, and consists of wave-mixing with a linearly-chirped pump yielding a converted waveform that is nearly equivalent to the signal waveform with a linear frequency chirp or equivalently a quadratic phase shift as required for a time-lens. Parametric time-lenses have phase-shifts in excess of 100π, which is significantly larger than the 10π maximally possible using an electro-optical phase modulator, and therefore greatly extend the applications of temporal imaging systems.
A drawback of using the sum-frequency-generation and difference-frequency-generation second-order nonlinear processes is that only a narrow range of materials possess a second-order nonlinear moment, and the converted waveform is inherently generated at widely different wavelengths from that of the pump or input signal. Waveform measurement based on temporal magnification using difference-frequency generation has yielded promising results, including single-shot measurement of ultrafast waveforms with a resolution of less than 900 fs for a simultaneous record length of 100 ps. Waveform measurements based on time-to-frequency conversion using electro-optic modulation have demonstrated a resolution of 3 ps over a 31-ps record length using multiple-shot averaging.
Here we demonstrate a parametric time-lens based on the third-order nonlinear process of four-wave mixing (FWM), and apply this time-lens to the creation of a silicon-chip-based ultrafast optical oscilloscope. As our device is based on the third-order Kerr nonlinearity, the FWM-based time-lens can be implemented in any material platform, including the CMOS-compatible silicon-on-insulator (SOI) photonic platform used here.
The output of this time-lens is generated at a wavelength close to those of the pump and input waves, enabling all the interacting waves to be in the S, C and L telecommunications bands, for example, which allows for the manipulation of all the waves using the well-established instrumentation and components available for these bands. Using our device, we perform measurements of highly complex waveforms with 220-fs resolution over record lengths larger than 100 ps. The combination of this 220-fs resolution and greater than 100-ps record length represents the largest record-length-to-resolution ratio (>450) of any single-shot-capable waveform measurement technique for the picosecond time range.
Furthermore, unlike commonly used techniques such as frequency-resolved optical gating and spectral-phase interferometry for direct electric-field reconstruction, our implementation directly measures the temporal amplitude profile using no reconstruction algorithm, allowing for rapidly updateable single-shot measurements.